Contact lenses as a promising therapy
Aim: This paper aims to provide insights into the scientific research about contact lenses and their development, and information about their use as therapeutic tools for various eye diseases. We outline the particular physiology and anatomy of the human eye and the relation with the resulting therapeutic difficulties. We describe therapeutic contact lenses such as bandage lenses and drug-delivering lenses.
Material and Methods: We have performed a bibliographic research on relevant developments regarding contact lens biomaterials using the research portals Web of Science, MEDLINE and Google Scholar. Reports and publications on worldwide ocular diseases, global current market analyses in the field of eye drops, as well as publications on modern drug release methods for ocular systems contain relevant information on the topic.
Results: Contact lenses have undergone a constant development, making them a sucessful tool in the ophthalmology market. Therapeutic contact lenses used as bandage lenses are effective tools in ophthalmic surgery and therapy.
Conclusion: Novel therapeutic contact lenses used for ocular drug delivery significantly improve the bioavailability and thus perform better than conventional eye treatments.
Introduction
Therapeutic contact lenses are special lenses used by ophthalmologists. These include bandage lenses or drug-delivering devices for the targeted administration of medication. Bandage lenses are particularly soft and permeable to oxygen. They protect the cornea in the case of injuries or after eye surgery and support or accelerate the healing process. For many eye diseases, medical contact lenses (MCLs) can also release drugs into the eye and offer better therapeutic options than conventional methods such as eye drops. Contact lenses (CL) are made of biomaterials with excellent optical quality, optimised mechanical features and safety properties. Their therapeutic applications are becoming increasingly important in addition to their use as optical vision aids and as cosmetic aids to change eye colour or mask possible irregularities of the pupil and iris.
Contact lenses: development and materials
L. da Vinci's 1508 "Codex D" contained scientific drawings illustrating the magnifying effect of water;1 but it is perhaps exaggerated to attribute the invention of the first CL to him because of this. It was not until more than 300 years later, around 1820, that the British astronomer Sir J. Herschel sketched a lens adapted to the shape of the eye. However, the first real CL were made of glass in 1888, by the physician A. E. Fick and the optician E. Kalt.2 Due to the hardness of the material, they were quite uncomfortable and also unhealthy, as glass deprived the eye of oxygen and increased the risk of corneal infections and hypoxia.
It was not until the advent of plastics that CL development made a breakthrough. W. Feinbloom3 produced the first lenses from polymethyl methacrylate (PMMA) in the USA in 1936. Acrylic glass, known as Plexiglas®, is a biocompatible and highly transparent material and allows for easier handling and processing than glass. The PMMA lenses were much more comfortable than the glass lenses, but they were still too stiff and impermeable to gas. They were large and covered the whole eye.
In the 1950s, O. Wichterle discovered the monomer 2-hydroxyethyl methacrylate (HEMA) and used it to make the first hydrogel for soft CL around 1960.4, 5 Poly(2-hydroxyethyl methacrylate) (PHEMA) was cross-linked with ethylene glycol dimethacrylate (EGDMA). The hydrophilic polymer gave the lens material a high water content and good flexibility. These noticeable improvements increased the popularity of contact lenses in the ophthalmology market significantly. PHEMA was further improved by copolymerisation with other hydrophilic monomers such as N-vinylpyrrolidone (NVP), methacrylic acid (MAA) or N,N-dimethylacrylamide (DMAA), which added amine, carboxylic acid and hydroxyl groups and increased the water content of the hydrogels.
Advances in material science and medicine have enabled the continuous optimisation of CL, especially with regard to wearing comfort and oxygen permeability. The introduction of siloxane units into polymer frameworks, for example with 3-[tris-(trimethylsiloxy)-silyl]propyl methacrylate (Tris) or fluorosiloxanes, resulted in silicone hydrogels (Si-H) that combined gas permeability with hydrophilicity and enabled longer lens wear. The repeated parts of Polydimethylsiloxane (PDMS) also have the advantage of repelling protein deposits on the lens surface better than soft CLs made from HEMA hydrogels.6
Modern, rigid CLs are also silicone-based and highly permeable to gas. Although they are called rigid (Rigid Gas Permeable, RGP), they are more flexible than earlier lenses made exclusively of PMMA. Polyvinyl alcohol (PVA) was also used to make hydrophilic lenses in the 1990s.7 Coatings with polymers such as polyethylene glycol (PEG) can also improve the wettability of intrinsically hydrophobic silicone materials.8 CL materials based on HEMA and silicone hydrogels can be further modified by grafting or incorporating biocompatible components such as hyaluronic acid (HA), chitosan, β-cyclodextrin or cellulose. A relatively new technique is to combine two gels resulting in double cross-linked or interpenetrating hydrogels. Polyvinylpyrrolidone (PVP),9 HA, chitosan, derivatised PEG, poly-2-hydroxyethyl acrylate (PHEA) and polyacrylamide (PAM) are some gel-forming substances that have been successfully intermixed and linked with PHEMA-based hydrogels to obtain double-networks with other functionalities.
In summary, CL materials today consist of copolymers that offer tailor-made properties, have optimal water content and good oxygen permeability, allowing for increased wearing comfort.
The problem of protein adhesion
However, as with all biomaterials that come into contact with biological tissues and fluids, CL material surfaces are often affected by protein deposition. The tear film alone contains many proteins secreted mainly by the lacrimal gland, ocular surface epithelia and conjunctival blood vessels. These proteins perform important protective functions throughout the eye, such as lubrication, antioxidant defence and supply nutrients to the ocular tissues.10 Over 490 different proteins have been identified in tear fluids, including hydrolases, protease inhibitors and proteins involved in the immune response and defence against oxidative stress. Lysozyme, lactoferrin, secretory immunoglobulin A, serum albumin, lipocalin and lipophilin make up the majority of tear proteins. Unfortunately, they can also contribute to incompatibilities when they interact with CL biomaterials. Protein adhesion can affect the optical properties of lenses, as well as negatively impact the oxygen permeability and wettability of the materials they are made of.11 Furthermore, layers of biomolecules such as lipids and proteins favour the attraction and accumulation of cells and bacteria on CL surfaces, increasing the risk of inflammation. Both hydrophilic and hydrophobic materials are affected by deposits.
HEMA hydrogels are most affected by protein deposition due to electrostatic attraction to positively charged molecules such as lysozymes.12 Consequently, comonomers such as NVP and DMMA further exacerbate this problem. Si-H exhibit lower rates of protein deposition but have a greater affinity for denatured proteins and lipids. Lipophilic PDMS literally dissolves lipids, which deposit on and even in the core of the siloxane matrix.
Many attempts have been made to reduce protein deposition on lenses, including polymer modifications. PHEMA hydrogels cross-linked with a small amount of HA, for example, were less susceptible to lysozyme absorption, while HA continued to improve the wettability of the material.13 A layer of 2-methacryloyloxyethyl phosphorylcholine (MPC) on the base substrate of a Si-H material inhibited lipid deposition and cell adhesion, producing a bio-inspired antifouling Si-H CL.
Threatening visual impairment: the human and economic burden
According to the WHO World report on vision,14 2.2 billion people worldwide suffered from blindness and visual impairment in 2019. More than 1 billion of these cases would have been preventable or curable. Most of these patients were older than 50 years old. Globally, the leading causes of blindness and visual impairment are conditions such as diabetic retinopathy (DR), eye traumata, age-related macular degeneration (AMD), cataracts, corneal infections from trachoma and glaucoma. The most common causes of impaired vision were cataracts (9.4%), uncorrected refractive errors (8.84%), glaucoma and corneal opacities (0.77% each), DR (0.39%) and trachoma (0.2%). AMD is one of the leading causes of blindness in older people worldwide: it is estimated that 196 million people globally were affected in 2020.15, 16, 17 The risks of visual impairment and blindness increase as demographics continue to grow and the population ages. Many age-related diseases such as AMD, DR and dry eye syndrome (sicca syndrome or keratoconjunctivitis sicca, KCS) need urgent and effective treatment.
Eye drops and lubricants are widely used for the treatment of various eye diseases and disorders, whether by prescription or self-medication. A global economic analysis estimated the eye drops and lubricants market size to be $22.63 billion by 2025, growing at a CAGR of around 4.7% (2018-2025). The analysis of type segments clearly confirmed the dominant market position of the artificial tears segment, followed by the hormones segment.18 As a result, the cost of medicines and treatments is steadily increasing and is a major economic burden for society. To better prevent visual impairment and treat eye diseases more effectively, modern therapies need to be further developed and existing ones improved.
The special anatomy and physiology of the eye
The human eye is poetically called the window to the world. To protect it from external dangers and ward off toxic substances, this complex optical system and at the same time sensitive organ is equipped with sophisticated protective mechanisms (Figure 1). The conjunctiva is a mucous membrane that covers part of the anterior sclera and the inner layer of the eyelids. It promotes moisture on the surface of the eye, provides the eye with nutrients and protects it from dust and dehydration. Together with the constantly renewing tear film, it protects the eyeball from friction and lubricates it. The conjunctival sac and cornea seal the outer surface of the eye and form the conjunctival and corneal eye barriers against particles and microorganisms.
The blood-ocular barrier, in turn, forms a comprehensive shielding system consisting of two elements: the blood-aqueous barrier19 and the blood-retinal barrier.20 The blood-aqueous barrier is the anterior barrier of the eye formed by tight epithelial cell contacts, known as tight junctions, between the endothelial cells of the iris blood vessels and those of the inner, non-pigmented ciliary epithelium. It separates the blood vessels from the aqueous humour and prevents it from clouding. Large molecules cannot pass through the barriers of the blood vessels in the iris. Special fenestrated blood vessels in the ciliary body, on the other hand, can supply the stroma, the middle and thickest layer of the cornea, with plasma proteins and molecules because they are permeable to these molecules. The blood-retinal barrier, in turn, controls the transfer of substances such as proteins, ions or water into and out of the retina through an inner and an outer physiological barrier. Here, too, tight junctions form interconnected diffusion barriers: between retinal capillary endothelial cells on the inside and between retinal pigment epithelial (RPE) cells on the outside. They separate the retina and vitreous body from the blood vessels, thereby enabling the immune privilege of the interior of the eye. The blood-ocular barrier prevents toxic or even lethal substances from entering the interior of the eye, maintains the homeostasis of the eye area and preserves vision.
These biological protective mechanisms simultaneously pose a major challenge to the administration of drugs into the anterior and posterior segments of the eye, as they present major obstacles to both topical and systemic applications.21 Rapid tear renewal and nasolacrimal drainage wash away eye drops within only a few seconds after instillation, and the blink reflex further impedes the adherence and retention of drugs on the ocular surface. Therefore, 90% of ophthalmic formulations based on topical drug delivery fail because of poor drug bioavailability due to precorneal elimination. The administration of drugs in the form of eye drops or ointments must therefore be frequently renewed, often leading to systemic side effects, due to non-adherence to therapy or even overdose. Drugs administered via intravenous injections and oral administrations can reach the choroid via the blood vessels, but the outer blood-retinal barrier hinders their further penetration, so that only 1-2% of the administered agents reach their therapeutic target.22 For the treatment of retinal diseases such as AMD, intravitreal injections are therefore often necessary to deliver a drug directly into the vitreous cavity. Conventional delivery systems thus fail to adequately supply both the outer and inner eye areas. However, new methods can improve the bioavailability and therapeutic efficacy of the required medication and contribute to a reduction in drug costs.23
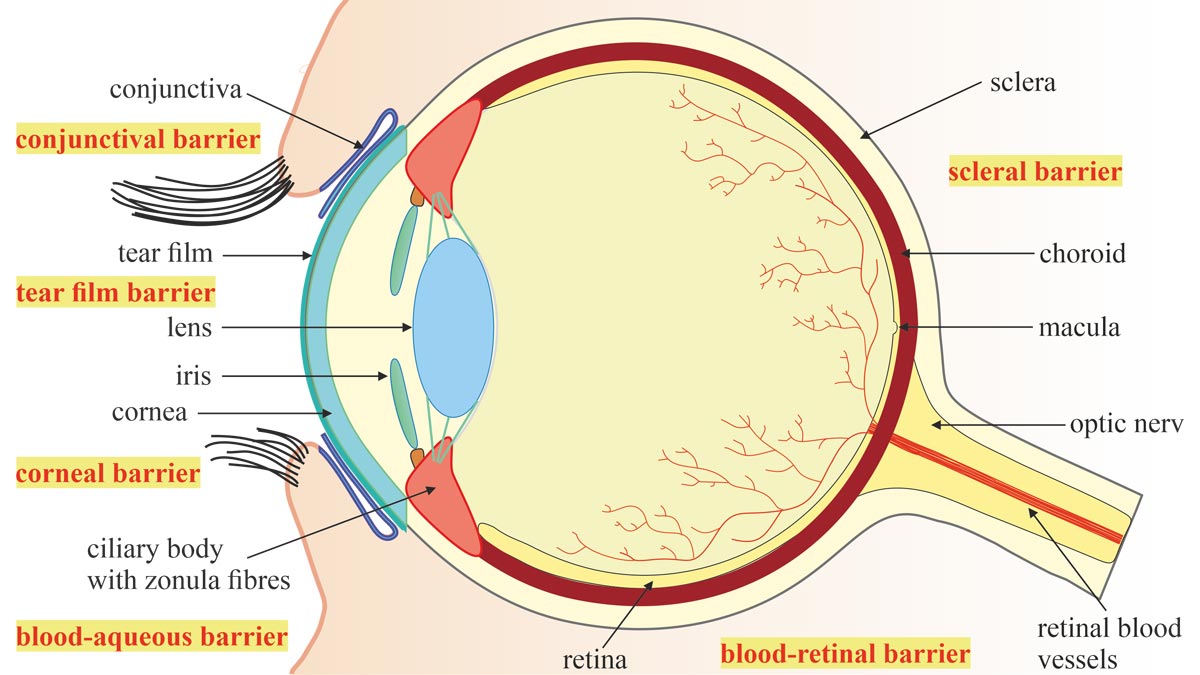
Therapeutic contact lenses and bandage lenses
Once developed purely as a visual aid to compensate for defective vision, the therapeutic potential of CLs quickly gained importance. Therapeutic contact lenses (TCLs) found early application in wound healing and in the treatment of corneal and ocular surface disease (OSD) caused, for example, by sicca syndrome or immunological disorders.24 TCLs can help reduce pain, improve comfort, promote healing and aid recovery. Various types of CLs and scleral lenses, which are mounted on the sclera instead of the cornea, are very suitable as so-called bandage lenses for the treatment of OSD and can be made of either hydrogel, PDMS hydrogel or collagen pads.
X. Galezowski,25 a French-Polish ophthalmologist (1832-1907), first reported the successful use of a bandage lens after cataract surgery in a publication in 1886. His bandage consisted of gelatine flakes impregnated with mercury(II) chloride as an antiseptic and cocaine as an anaesthetic. When PHEMA-CLs hit the market in the 1970s, their hydrophilic properties, elasticity and softness made them ideal for therapeutic use.26 They relieved corneal discomfort after surgery and protected the corneal epithelium from abrasion caused by blinking and eye movement. Close-fitting lenses were also able to fix the epithelium against Bowman's layer, improving the healing process. The only CL approved by the US Food and Drug Administration (FDA) for therapeutic use in corneal disease was the Warner-Lambert Softcon® in 1974. However, Bausch and Lomb's Soflens® was also used for this purpose.27, 28 Both were made of PHEMA cross-linked with EGDMA, were large in diameter (12.5-15 mm) and had a water content of approximately 39% and 55% respectively when hydrated. Both have proven to be helpful in cases of bullous keratopathy, exposure keratopathies and corneal dystrophy.
Therapeutic soft CLs were rapidly proving effective for the treatment of many different conditions such as recurrent corneal erosions, KCS, keratoconus, post-traumatic aphakia, neurotrophic and lagophthalmic keratitis, ocular pemphigoid, Stevens-Johnson syndrome, etc.29 Bandage CLs (BCLs) are now replacing wound ointment dressings which were once an integral part of the treatment of ocular diseases and injuries. The reasons for this development are based on the effectiveness of high-quality BCLs in protecting the eye, relieving pain, supporting the healing process and, last but not least, in delivering therapeutic agents when also used as drug carriers. Furthermore, they enable patients to resume their daily tasks better and faster than ointment dressings do during the usually lengthy therapies.
Wound dressings have the serious disadvantage that they seal the eye and keep it closed for a long time. However, corneas depend on the supply of oxygen (O2) from atmospheric air. When the eyes are closed, for example during sleep, a limited O2 supply is taken over by the blood vessels of the eyeball and the aqueous humour. This results in a significant drop in O2 partial pressure and thus in relative hypoxia.30 As a result, even a healthy cornea swells by 3-4% during sleep (corneal oedema). An injured and covered cornea therefore suffers all the more from an O2 deficiency, and corneal oedema makes it even more vulnerable and susceptible to infections. O2 permeability is a very important factor in supporting the healing process, especially with long-term bandage lens wear. O2 permeability is expressed in units of Dk: D is the velocity of O2 through the material [cm2/s] and k is the O2 solubility in the material [ml O2/ml x mm Hg]. The problem can be overcome by selecting materials for BCLs that have the highest gas permeability, e.g., Si-H. Soft CLs are often the best choice for dressings as they irritate the anterior part of the eye less than rigid dressings. Hydrophilic CLs such as PHEMA-based lenses are soft and can also be easily loaded with therapeutic agents, e.g., antibiotics. However, accumulation of toxic drug concentrations in the post-lens tear lake and on the cornea must be avoided. Dehydration rates of hydrophilic CLs must also be considered as they may limit their use. Dehydration depends on the material, lens thickness and environmental conditions.
BCLs and especially soft CLs made of Si-H for extended wear are recommended for various applications to promote wound healing after trophic ulcers and refractive surgery, to provide corneal protection and sealing and to relieve ocular pain, e.g., corneal injuries and dystrophies. BCLs are therefore used:
- to improve healing after keratoplasties, trabeculectomies and amniotic membrane transplants;
- to protect the cornea and prevent complications such as infections, epithelial defects and stromal melting after implantation of a Boston keratoprosthesis (KPro);31
- to seal small corneal perforations or as a bridging treatment before penetrating keratoplasty;
- to improve epithelial healing and relieve pain after trauma or surgery, and for recurrent epithelial defects;32
- to minimise hypoxia in patients who need to wear bandage lenses for prolonged periods. In difficult post-traumatic cases of recurrent corneal erosions, the use of Si-H-based CLs can last up to 3 months, but they must be replaced every 2 weeks;33
- for pain relief and support of the healing process in the therapy of bullous keratopathy and filamentous keratitis;
- to promote corneal healing in serious conditions such as severe KCS, Stevens-Johnson syndrome or toxic epidermal necrolysis (preferably with highly O2-permeable scleral or semiscleral lenses);
- to protect the cornea as a mechanical barrier in patients suffering from eyelid problems such as trichiasis, ptosis and conjunctival scarring;
- after refractive procedures such as laser-assisted in situ keratomileusis (LASIK) or subepithelial laser keratomileusis;
- to promote re-epithelialisation after photorefractive keratectomy (PRK). The review of 13 studies evaluated the pain-relieving results of bandage lenses applied immediately after PRK in 842 patients. Early postoperative outcomes of two types of bandage lenses showed a reduction in pain scores and a decrease in defect size in the epithelialised area one day after surgery;34 and
- to attenuate unpleasant sensations after the application of cyanoacrylate adhesives.35
Contraindications for therapeutic lenses and bandage lenses
TCLs are quite effective in the treatment of corneal and ocular surface diseases, but there are of course some contraindications and possible complications:
- TCL use may be contraindicated in cases of KCS, infectious keratitis, corneal anaesthesia, neurotrophic or significant exposure keratopathy with defective eyelid position or movement.
- Prolonged wear of the CLs may cause infectious keratitis and corneal hypoxia, which may, in turn, result in corneal oedema, neovascularisation and allergic conjunctivitis. Therefore, prophylactic medication should be administered.
- Common complications of TCL use are often associated with predisposing corneal diseases such as corneal oedema, microbial keratitis and neovascularisation. Virulent organisms may also infect TCL wearers more frequently.36
It is therefore necessary to avoid any risk of infection and to limit contamination of the TCLs as much as possible, for example by minimising their manipulation or shielding the CL-bearing eye. Bandage lenses should also be renewed frequently and treatment for ocular wetting should be continuous. However, many patients are reluctant to wear a bandage lens after traumatic surgery, and some of them cannot tolerate it at all. Drug-delivering TCLs offer additional benefits to patients. This is where modern material development plays a crucial role.
Contact lenses as ocular drug-delivery systems
Injuries, chemical erosion, burns as well as infections and corneal diseases urgently require pain-relieving and drug-releasing therapies. Bandage lenses to protect diseased or injured eyes are therefore usually loaded with therapeutic drugs and used for ocular drug delivery (ODD). Some CLs have been specifically designed to improve ODD to overcome the ocular barrier, improve ocular bioavailability of therapeutic drugs and maintain the required drug concentrations in the eye.37 Novel biopharmaceutical methods have already improved the efficacy of ocular drug-delivery systems (ODDS) through the use of CLs (Figure 2),38 gently delivering the necessary drugs to the ocular region over a longer period of time while reducing the risks of systemic side effects.
Unfortunately, the two major problems of CLs as a means for ODD are the limitation of their loading capacity on the one hand and the early, sudden release of drugs on the other. Ideally, drug release should be controlled and sustained, preferably at a constant rate, i.e., following zero-order release kinetics. Several techniques have been developed in order to achieve these goals and to address the challenge of the most sustained drug release possible.
Materials and methods
Materials and physical properties of CLs must first be carefully considered to optimise drug uptake and release and to meet the physiological requirements of the ocular tissues and the needs of the particular medical treatment. The most commonly used materials for drug delivery are soft CLs made of PHEMA and Si-H. However, the polymers need to be further improved to increase their efficiency. This can be done using many different methods such as copolymerisation, polymer modification by grafting or coating or molecular architecture design such as molecular imprinting (MI).
Soak and release is a common and simple method of introducing drugs into soft CLs.39, 40 The dissolved pharmaceuticals penetrate the hydrogel network and are released onto the ocular surface after lens insertion. Hydrogel lenses can easily absorb dissolved drugs thanks to their 3-D network and good swelling ability. Even hydrophobic drugs, small molecules and nanomaterials can be absorbed and retained in their network. This method proved suitable for many hydrophilic and hydrophobic ophthalmic drugs, including moxifloxacin, diclofenac and ketorolac, levofloxacin, chlorhexidine, timolol maleate, HA, vancomycin HCl or polymyxin B sulphate. Such drug-delivering CLs have already demonstrated better performance than conventional eye drops.
Atropine-releasing CLs could provide pharmacotherapeutic management of paediatric myopia progression with improved ocular bioavailability and avoidance of the adverse effects of atropine drops. The prevalence of myopia in children is increasing worldwide, especially in East Asia. High myopia entails also an increased risk factor for degenerative eye diseases in old age. Low-dose atropine eye drops have already shown good results in studies to curb the progression of myopia. However, the mechanisms by which the antagonist of the muscarinic receptor inhibits myopia development are not yet fully understood.41 In Asia, atropine is already widely used for myopia control, but so far there is no drug approval for atropine drops for this specific use in Germany and no FDA approval in the USA. Currently, the treatment with atropine continues to be under scrutiny. In any case, the distressing side effects of the antimuscarinic drug, including prolonged pupillary mydriasis, reduced visual acuity and inhibition of accommodation, only permit the administration of low concentrations of atropine. Treatment with 0.10 to 0.01% eye drops is sometimes carried out in combination with multifocal and/or orthokeratological CLs (Ortho-contact lenses) to correct refractive errors.
Commercial CLs made of various materials were studied for their uptake and delivery capacities of two antimuscarinics, atropine sulphate and pirenzepine dihydrochloride.42 The CLs were swollen in 1-10 mg/ml solutions and their drug release within 24 hours was studied in vitro. Most of the release of the pharmacological agents was very quick (within the first 20-30 min), which would limit long-term administration. The water content and ionic charges of the materials played the biggest role here, Si-H were less suitable than other hydrogels in this study. The use of CLs as an ODD for atropine was also experimentally tested on Si-H lenses of different compositions.43 Atropine could be taken up both during lens swelling and during Si-H synthesis without compromising critical lens properties such as water uptake, light transmission and surface hydrophilicity. The hydrophilicity of the lens material also positively influenced uptake and delivery in this case. A sustained release over more than 14 days was observed.
The controlled administration of low doses of atropine via CL release could be used effectively against myopia progression in combination with orthokeratology in the future.
In general, CLs prepared by the swelling method often show poor uptake of the drug and initial excessive release followed by subtherapeutic concentrations. In addition, they often carry the risk of altering critical lens parameters.
Drug-loaded niosomes were used to improve drug loading and release capacity.44 Niosomes are non-ionogenic, surfactant-based vesicular systems suitable for the controlled delivery of drugs that can be absorbed into their interiors.
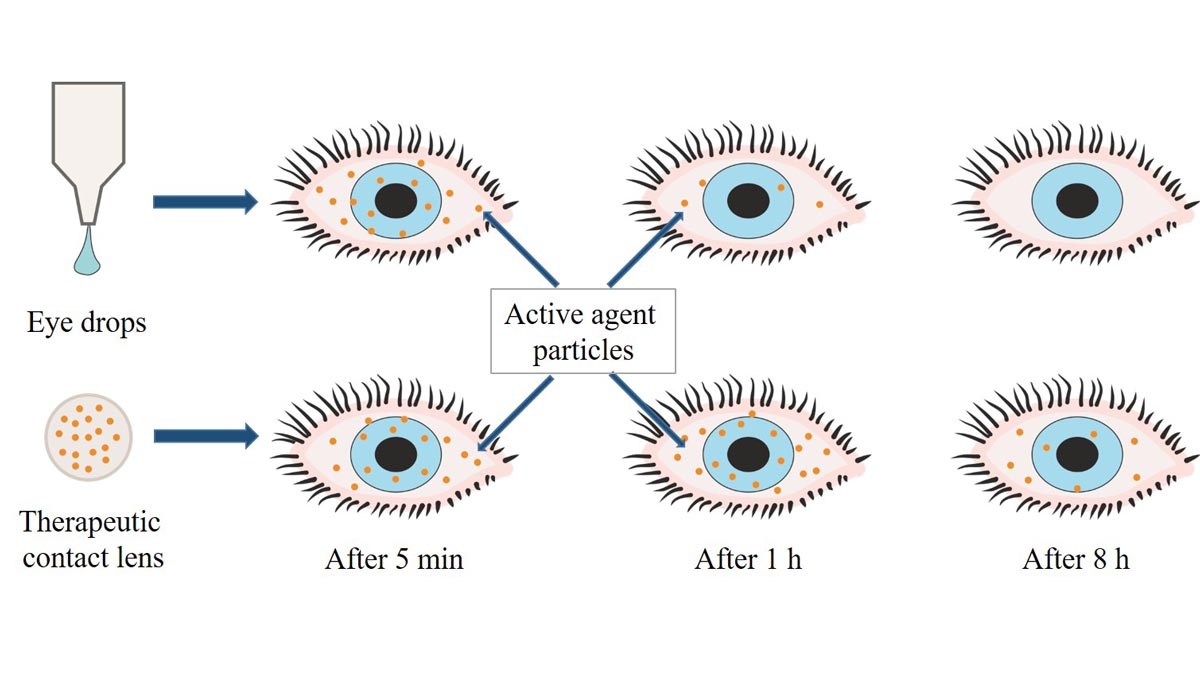
Ofloxacin, an antibiotic for ocular infections such as conjunctivitis, could be integrated into niosomes, and CLs made of HEMA/PDMS were subsequently swelled in niosome solutions loaded with ofloxacin. This increased the uptake capacity of the drug in the lenses and achieved a more sustained release without compromising their opto-physical properties. They performed better than conventionally impregnated lenses and showed a better healing effect than treatment with eye drops. Similarly, soft CLs after swelling with timolol-loaded nanostructured lipid carriers (NLCs) showed high uptake and release rates and good clinical outcomes in glaucoma patients.45
An alternative way to incorporate drugs into polymer matrices of CLs is to introduce nanoparticles (NPs), which in turn encapsulate active agents. Encapsulation in NPs has confirmed the antimicrobial potential of silver to control microbial growth and colonisation of CLs.46 In this in vitro study, hydrogel CLs were first swollen in suspensions of colloidal silver NPs. The subsequent silver release reduced the adhesion of Staphylococcus aureus (S. aureus) and Pseudomonas aeruginosa (P. aeruginosa) to the lenses. It also caused a decrease in the number of colony-forming units of Acanthamoeba castellanii (A. castellanii) and a reduction in the viability of their trophozoites.
Various new and patient-friendly formulations using nanotechnology-based delivery strategies have been developed for the therapy of eye diseases. Nanotechnology-based systems are designed to overcome ocular barriers and interact with specific ocular tissues. Molecules have been designed to work with nanocarriers, for example, to treat glaucoma, retinal and choroidal diseases. Polymer and lipid NPs, as well as liposomes, are known to be able to entrap many types of molecules. Once embedded in CL matrices, the NPs release their charge, which first diffuses through the hydrogel of the lenses and eventually reaches the ocular surface.
There are mainly two ways to incorporate NPs into polymers: by coating the final polymer material or by adding them directly into the monomer mixture in the pre-polymerisation step.
Using the principle of the latter method, e.g., PHEMA lenses were enriched with NPs containing dexamethasone sodium phosphate (DXP) and cyclosporine. The anti-inflammatory, immunosuppressive agent DXP could be loaded into chitosan-NPs first obtained by ionic gelation.47 Cyclosporine, a hydrophobic molecule used to treat KCS, could be encapsulated in micelles of cholesterol hyaluronate.48 The loaded particles were added to the monomers prior to photopolymerisation. In vitro tests of the resulting hydrogel matrix demonstrated consistent drug delivery with good release rates and no adverse changes in the optical or morphological properties of the final lenses.
CLs loaded with plasma-activated water are a special case of the uptake method.
An antibiotic-free alternative for the treatment of corneal infections has been developed as a new therapeutic concept in ophthalmology. Worldwide, keratitis is one of the main causes of blindness and it is very difficult to treat it effectively. It is very painful and usually leads to incurable corneal opacity and loss of vision. In microbial keratitis, pathogens such as bacteria, viruses, fungi and Acanthamoeba are responsible for inflammation and disease. “Free-living amoebae” are common protozoa that can infect humans. Amoebic keratitis (AK) primarily affects lens wearers via contaminated contact lenses, improperly disinfected containers or inadequately cleaned hands before handling contact lenses. Conventional treatments include disinfectants and antibiotics, but these can often cause unwanted side effects, including severe corneal damage.
Plasma medicine belongs to a relatively new therapeutic area, but it has already achieved clinical progress in the treatment of chronic wounds and severe infections, even with resistant microbes, in dermatology, dentistry, oral medicine and orthodontics. Plasma is used for sterilisation of surfaces and wounds, as well as for haemostasis and wound healing. Its use for the medical treatment of cancerous human tissue is still being evaluated. A new approach to combat corneal infections caused by Acanthamoeba using plasma technology has been investigated.
Cold atmospheric plasma is a partially ionised gas that generates UV radiation, heat and various reactive particles such as electrons, ions, excited atoms and molecules, and thus has an antimicrobial effect.49 The bactericidal effect of direct irradiation of bacterial colonies of Staphylococcus aureus and Pseudomonas aeruginosa with cold plasma has been successfully demonstrated.50, 51 Water exposed to plasma irradiation showed the same effect indirectly (Figure 3).
The so-called fourth state of aggregation leads to the formation of reactive oxygen (ROS) and nitrogen (RNS) species: hydroxyl radicals •OH, atomic O, ozone O3 and peroxides such as H2O2 as well as NOx, NO2- and NO3- penetrate the water and dissolve in it. The water is thus activated, enriched and acidified. The significant germicidal effect results from the oxidation-reduction potential (ORP) of H2O2 and NO3- and the high reactivity of the oxygen and nitrogen species (RONS). In addition, binding reaction products from NO2- with peroxides, synergistic effects of acidic pH with anionic nitrogen oxides as well as interactions of photons from the visible and UV spectrum with the various reactive species have shown a significant germicidal effect. All these highly reactive species induce oxidative stress in microbes leading to the destruction of their cell membranes.
Si-H CLs swollen in plasma-activated water (PAW) acquired this plasma-induced bactericidal effect. In vitro experiments confirmed the potential of such CLs to destroy the cell membranes of amoebae and inactivate them. Further studies will explore the potential applications of this process.
The modification of polymeric hydrogels, for example by copolymerisation with suitable monomers, is another approach for the development of networks with the ability to absorb and release drugs. For example, the addition of ionic monomers during polymerisation creates binding sites for ionic molecules. MAA is one of these useful comonomers with anionic character, often used in the production of hydrogels for drug release.
Copolymerisation of a polymerisable derivative of β-cyclodextrin (β-CD) with HEMA resulted in PHEMA-co-β-CD hydrogels. Complexation of drugs such as hydrocortisone and acetazolamide within the lipophilic interior of the CD unit formed by the 7-membered ring of glucose building blocks thus allowed sustained drug release over several days.52
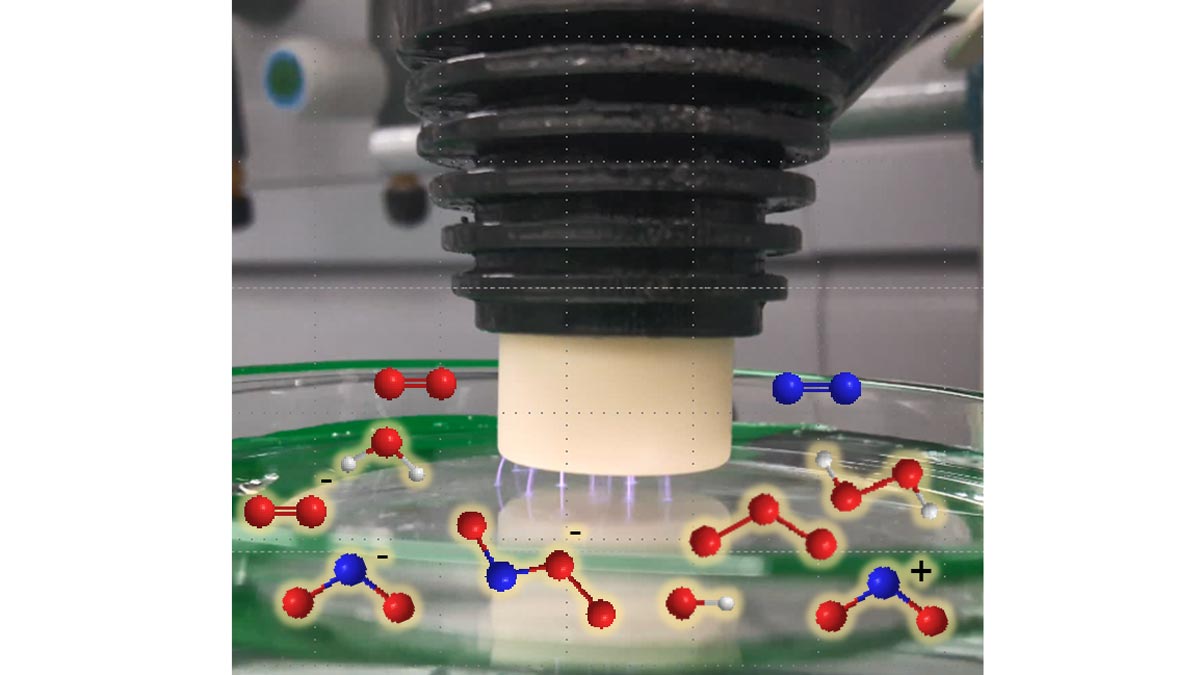
Thin films of the biodegradable, biocompatible and FDA-approved copolymer poly(lactic-co-glycolic acid) (PLGA) were prepared together with ciprofloxacin (CFX) by solvent casting and then coated with PHEMA. This resulted in a CL prototype that released the drug at therapeutically relevant and sustained rates and was effective in inhibiting CFX-susceptible Staphylococcus aureus.53 Similarly, latanoprost was integrated into PLGA films, which in turn were embedded in a PHEMA matrix. The developed drug-delivering CL continuously released the glaucoma drug within about one month.54
Molecular imprinting technology (MIT) consists of a multi-step synthesis of a highly cross-linked polymer matrix into which target molecules are embedded in prefabricated cavities.
G. Wulff55 first applied MIT to synthetic polymers in 1972. Since then, this technology has found numerous applications in areas such as chromatography, catalysis, chemical and biological sensor technology and biotechnology. Due to the lock-and-key principle, molecularly imprinted polymers (MIPs) are also suitable for immunoassays, artificial antibodies and drug-delivery systems.
It requires at least one molecule as a template, a functional monomer and a crosslinker to create a molecularly imprinted polymer (MIP) with affinity sites for specific molecules. In the simplest case, the template molecule or imprint is first complexed with a functional monomer. The template-monomer complex can be controlled by covalent or non-covalent interactions such as ionic interactions or hydrogen bonds. MAA is often used as a functional monomer because it can act as both a donor and acceptor of hydrogen bonds. The subsequent copolymerisation of the functional monomer with a large amount of crosslinker produces a surrounding matrix that gives the network good stability. Due to self-assembly mechanisms, the polymer chains then form around the imprint.56 MIP usually contain additional monomers as well as a porogenic solvent in their matrix. In CL materials, copolymerisation occurs with common monomers such as HEMA, NVP, Tris or DMAA.
Non-covalent bonds can be easily washed out, leaving pre-formed, structured cavities with chemical affinity for the template molecule, which can re-insert reversibly (Figure 4).
Structural optimisation of the ad-hoc cavities increases drug-delivery efficacy and regulates the release rate. Zero-order release kinetics and sustained release properties are greatly enhanced by tailored interactions between the embedded drug and the polymer matrix. Modulation of controlled release can also be driven by varying the ratio of functional monomer to template (M/T). This sophisticated and complex technology requires in-depth knowledge of the interactions between molecules. MIPs must comply with biocompatibility and physiological toxicity limits in the specific case of application as useful drug stores for ODDS. Therefore, a washing step is almost always required to eliminate toxic residues of the chemicals used in the polymerisation process. Since the target molecule is usually bound via non-covalent interactions, it is flushed out in the process and must be reloaded at the binding sites of the MIP.
Many examples of MIP-based ODDS have already demonstrated the potential of MIT for ocular therapy.57
The first imprinted CL contained timolol as a template with non-covalent interactions to the MIP. Imprinted hydrogels containing timolol maleate in a MAA-based system were prepared by photopolymerisation together with N,N-diethylacrylamide and EGDMA. After washing out timolol, the beta-blocker was loaded again. The MI method improved the loading capacity thanks to the increased affinity for timolol, and the drug release was both sustained and long-lasting.58
A template polymer system for soft daily CLs made from a copolymer of HEMA and diethylaminoethyl methacrylate was also used to control the release of non-steroidal anti-inflammatory sodium diclofenac (DCF). The affinity of the drug for the MI product was characterised by ionic interactions and the M/T ratio.59, 60
Efficient soft CLs made of PHEMA hydrogel using a MIT design were able to take up large amounts of the antibiotic thanks to the good binding interactions between norfloxacin (NRF) and acrylic acid (AA). Optimising the molar ratio of AA to NRF resulted in a release performance of more than 24 hours.61
Imprinted HEMA hydrogels with MAA as the functional monomer were also investigated as carriers of CFX.62 High ratios of MAA to CFX improved the binding properties and affinity for CFX and allowed for a good, controlled release. The in vitro study demonstrated the antibacterial efficacy of CFX-loaded MIP hydrogels against Pseudomonas aeruginosa (P. aeruginosa) and Staphylococcus aureus (S. aureus).
A biomimetic approach achieved the controlled release of HA from imprinted, hydrogel CLs. The biopolymer HA is a non-sulphated glycosaminoglycan with a high molecular weight and is part of the extracellular matrix. HA is commonly used in artificial tear products and is beneficial for the treatment of ocular discomfort and KCS thanks to its lubricating properties. In this bio-inspired MI strategy, monomers were selected that have a similar binding affinity as biological receptors towards HA: Acrylamide (AM) with an amide group, N-vinylpyrrolidone (NVP) as a 5-membered lactam and diethylaminoethyl methacrylate (DEAEM) as a cationic acrylate. Thanks to the strong affinity of HA for the hydrogel, its diffusion was slow, and HA could be delivered at a therapeutic rate for 24 hours in a controlled manner.63
Applied to CL materials, molecular imprinting of polymers thus allows better drug upload and sustained release over a longer period of time, so that daily and even prolonged wear of CLs as an ODD can make a significant contribution to the therapy of ocular diseases.
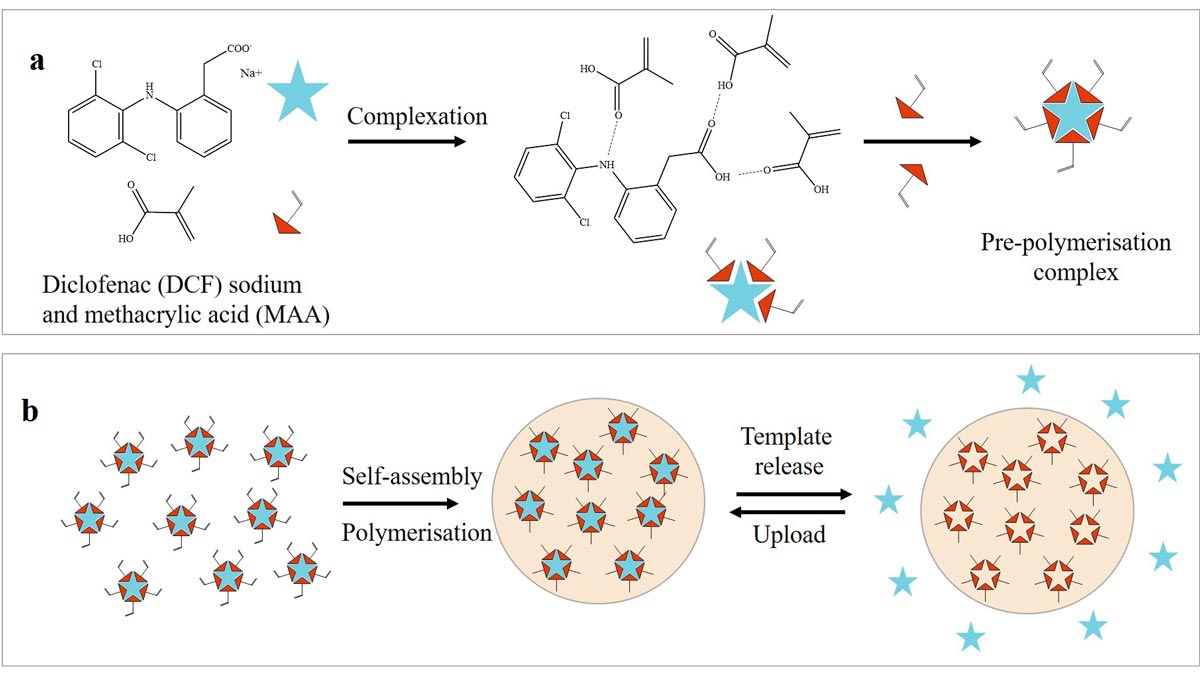
Conclusions
CLs have proven to be therapeutic devices with positive effects in many ways. Bandage lenses are part of ophthalmic therapy as long-established, indispensable, pain-relieving and curative aids.
In order to be used as ODDS, TCLs must also fulfil all the important characteristics of CLs, meaning that the introduction of drug molecules or NPs must not affect the water content, oxygen and ion permeability, and comfort. Limiting protein adhesion and preserving tribological and mechanical properties must also be considered for high patient compliance.
Last but not least, optical clarity and a light transmission of at least 90% should be maintained and the refractive index should correspond to that of the cornea (about 1.37).
The use of CLs as ODDS can increase the ocular bioavailability of drugs and thus improve ocular therapies.
Nevertheless, material processes and performance need to be further researched and optimised.
In this regard, the production of sophisticated materials is lengthy and expensive. There is therefore still a long way to go before they can be perfected and made available to a broad market with such high patient demand.
Conflict of interest
The authors declare that there is no conflict of interest.
.
.
Abbreviations
AA: acrylic acid
AK: amoebic keratitis
AM: acrylamide
AMD: age-related macular degeneration
BCL: bandage contact lens
β-CD: β-cyclodextrin
CFX: ciprofloxacin
DCF: sodium diclofenac
DEAEM: diethylaminoethyl methacrylate
DMAA: N,N-dimethylacrylamide
DR: diabetic retinopathy
DXP: dexamethasone sodium phosphate
EGDMA: ethylene glycol dimethacrylate
FDA: Food and Drug Administration
HA: hyaluronic acid
HEMA: 2-hydroxyethyl methacrylate
KCS: keratoconjunctivitis sicca (dry eye syndrome or sicca syndrome)
CL: contact lens
KPro: keratoprosthesis
LASIK: Laser In Situ Keratomileusis procedure
MAA: methacrylic acid
MI: molecular imprinting
MIP: molecularly imprinted polymer
MIT: molecular imprinting technology
MPC: 2-methacryloyloxyethyl phosphorylcholine
NLC: nanostructured lipid carriers
NP: nanoparticles
NRF: norfloxacin
NVP: N-vinylpyrrolidone
ODD: ocular drug delivery
ODDS: ocular drug-delivery system
ORP: oxidation-reduction potential
OSD: ocular surface disease
PAM: polyacrylamide
PAW: plasma activated water
PDMS: polydimethylsiloxane
PEG: polyethylene glycol
PHEA: poly-2-hydroxyethlyacrylate
PHEMA: poly-2-hydroxyethyl methacrylate
PLGA: poly(lactide-co-glycolide)
PMMA: polymethyl methacrylate
PRK: photorefractive keratectomy
PVA: polyvinyl alcohol
PVP: polyvinylpyrrolidone
RONS: reactive oxygen and nitrogen species
RPE: retinal pigment epithelium
Si-H: silicone hydrogel
TCL: therapeutic contact lens
Tris: 3-[tris-(trimethylsiloxy)silyl]propyl methacrylate
COE Multiple Choice Questionnaire
The publication "Contact lenses as a promising therapy" has been approved as a COE continuing education article by the German Quality Association for Optometric Services (GOL). The deadline to answer the questions is 1st March 2024. Only one answer per question is correct. Successful completion requires answering four of the six questions.
You can take the continuing education exam while logged in.
Users who are not yet logged in can register for ocl-online free of charge here.