The Role of the Ciliary Body in Aqueous Humor Formation
Purpose: The purpose of this article is to summarize current thinking regarding the anatomy and physiology of aqueous humor production by the ciliary body.
Material and Methods: The article represents a synthesis of experimental work done by the author and by other investigators.
Results: The pars plicata region of the ciliary body is the sole source of aqueous humor production. An array of intramembranous ports, pumps and intercellular junctions move selected ions from the ciliary body stroma into the cleft between nonpigmented ciliary epithelial cells. The resulting hypertonicity draws water into that narrow cleft and, together with water channels, produces the major fluid component of aqueous humor.
Conclusions: The ciliary body operates similar to an exocrine gland of the “secretory sheet” category and produces aqueous humor using autonomic and humoral regulatory mechanisms.
Introduction
Aqueous humor (AH) is the constantly replenished nutrient fluid upon which the avascular tissues of the anterior segment of the eye depend. These tissues include the lens, cornea and trabecular meshwork. It is the balance between aqueous production and aqueous outflow that maintains intraocular pressure (IOP) at a relatively steady state. Maintaining this stable pressure keeps the various optical elements of the eye at relatively constant distances, in order to keep light in focus, but without rising to pressures that could damage the neurovascular elements of the retina and optic nerve. Intraocular pressure remains the only treatable risk factor for vision loss due to the various forms of glaucoma. Some of the pressure-reducing strategies in glaucoma management are aimed at decreasing aqueous production rather than augmenting aqueous outflow. Summarizing the anatomical, physiological and regulatory principles of aqueous humor production, as a foundation for understanding therapeutic strategies, is the purpose of this article.
Aqueous humor
Aqueous humor (AH) is a clear nutritive fluid that serves the metabolic needs of the avascular tissues of the ocular anterior segment. The protein content of aqueous humor is very low, presumably to reduce light scattering in the ocular media. Some of these proteins are locally made but most are plasma-derived. Many of the locally made proteins play a critical role in creating an immunosuppressive environment in the anterior chamber.1 These immunoregulatory proteins modify the kinds and types of immunoregulatory responses that can be mounted within the eye, since some of them could have more devastating effects on the delicate tissues of the eye and on vision than might occur by simply tolerating the presence of certain foreign antigens. One of the most interesting of these is transforming growth factor-beta (TGF-b), which fosters a pro-fibrotic environment when its levels are elevated. In this regard it is interesting to note that the levels of TGF-b are elevated in the AH of patients with primary open angle glaucoma (POAG).2 The ionic and nutritional constituents of AH are summarized in Table 1. Most of these constituents are present in amounts roughly proportional to their levels in plasma.3 The major exceptions to this are in levels of ascorbate, lactate, pH and total protein. Each is discussed below.
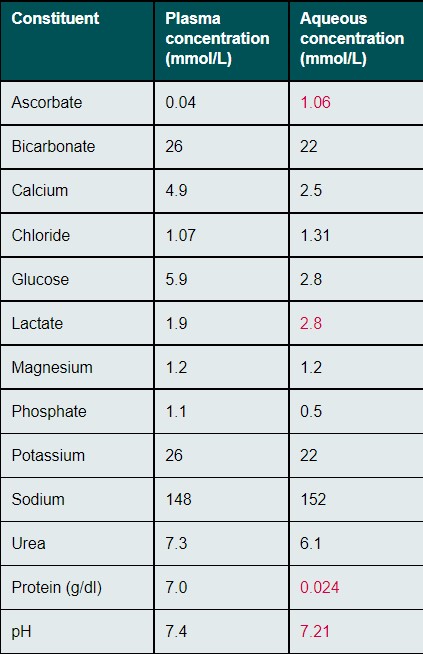
Ascorbate functions as an anti-oxidant and scavenger of superoxide radicals. Ascorbate interacts directly with UV light to prevent UV tissue damage. Interaction of ascorbate with UV light produces hydrogen peroxide, which is then reduced to harmless water by enzyme systems that are replenished within cells using energy obtained from glucose taken from AH.4 This added demand for glucose, to replenish reducing enzymes, plays a role in explaining why glucose levels are lower in AH than in plasma. Also playing a role is the fact that the tissues of the ocular anterior segment are forced to operate using anaerobic metabolism, due to the low oxygen levels. Thus, only two molecules of ATP are generated from a molecule of glucose compared to the usual 36 generated when oxygen levels are higher. Hence, more glucose must be metabolized to make up for the low oxygen levels in order to sustain the supply of ATP required as the main energy source of cellular activity. Thankfully, this situation is not worsened in diabetes because these avascular tissues do not require insulin in order for glucose to enter their cells. Lactate levels are higher in AH than in plasma. But the lactate in AH is not part of AH as it is produced. It is the end-product of the anaerobic metabolism employed by the avascular tissues bordering the low oxygen environment of the anterior chamber. Since complete turnover of the AH in the eye occurs every 90-100 min, the levels of lactate generally reflect the level of glucose metabolism during that time period. Ascorbate and Lactate are both acidic. It is largely their presence in the aqueous humor that renders the slightly lower pH in AH than that found in plasma. One of the biggest ways in which AH differs from plasma is in its protein concentration. The protein concentration of AH, in aliquots obtained from the anterior chamber, is less than 1% of that found in plasma (Ratio of aqueous/plasma = 0.024 / 7 gm/dl).5 Although the ratios between the various blood-derived proteins in AH generally reflect their relative concentrations in plasma, there are some clear exceptions.6 Among those found in higher concentration in AH, is transferrin, an iron-scavenging protein that reduces the risk of tissue damage from iron toxicity, mediated by a Fenton reaction, in the case of intraocular hemorrhage.7
While many AH proteins, especially those serving immunoregulatory roles, are locally produced, much of the protein in AH is derived from the bloodstream. Despite this, current evidence suggests that AH, as it is secreted into the posterior chamber, is plasma-protein free. It has been demonstrated that an anterior diffusional protein pathway exists in the normal eye that moves plasma proteins from a depot in the ciliary body stroma, through the uninterrupted stromal pathway to the root of the iris and then the anterior chamber, by-passing the posterior chamber entirely (Figure 1a).8-10 Proteins entering the anterior chamber along this pathway are prevented from returning to the posterior chamber by tight junctions that join the posterior epithelial cells of the iris.11 Via this anterior diffusional pathway from the ciliary body, the plasma protein content of the AH is supplemented just prior to the entry of AH into the outflow pathway (Figure 1b).
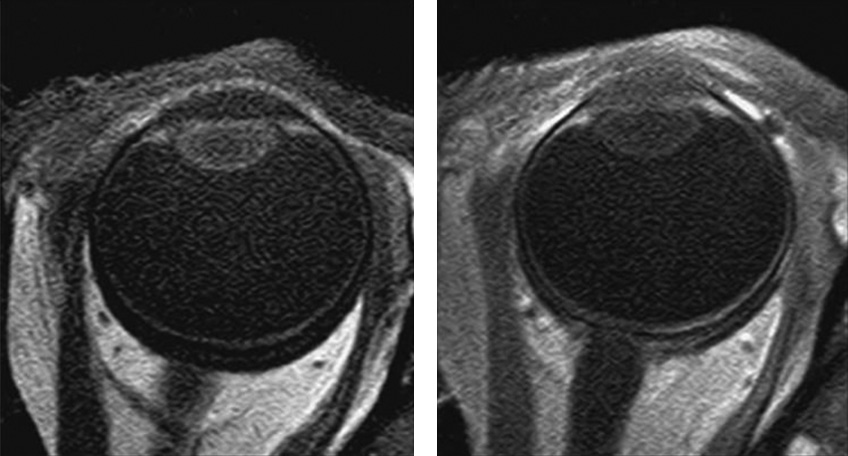
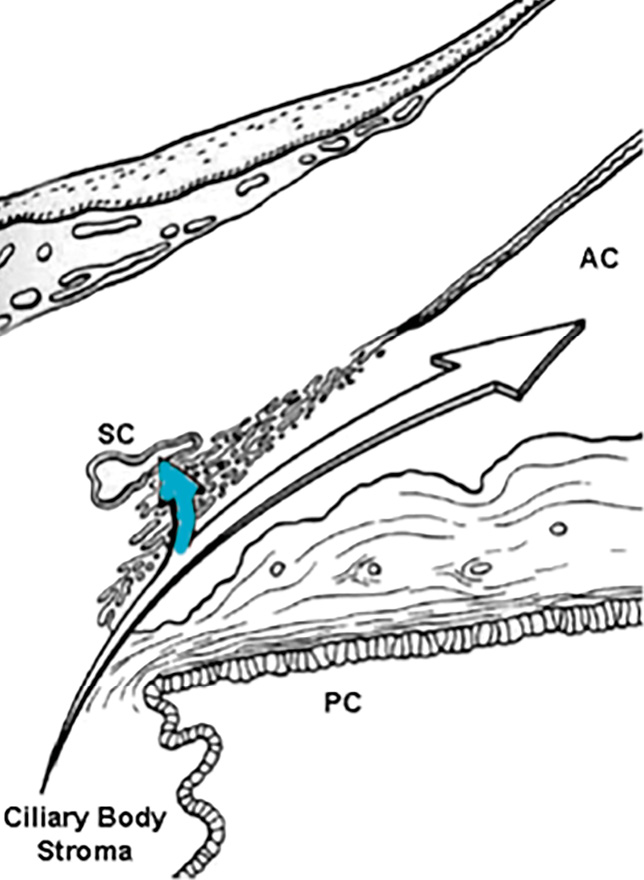
Aqueous humor circulation
Due to the presence of tight junctions between cells of the posterior iris epithelium, AH cannot pass through the iris. Thus, to pass from the posterior to the anterior chamber, AH must flow through the pupil. The pupillary margin rests on the anterior lens capsule, creating a one-way valve that prevents reflux of AH back into the posterior chamber. Changes in the anatomical relationship between the pupillary margin and anterior capsule of the lens, particularly with the pupil at mid-dilation, can resist the flow of AH into the anterior chamber (relative pupillary block). Similarly, inflammation-induced adhesions of the pupillary margin to the anterior surface of the lens (posterior synechiae) can obstruct aqueous flow. Both will elevate intraocular pressure. Once AH enters the anterior chamber, it circulates and is mixed in a convection current driven by the temperature difference between the warmer iris and the cooler cornea. AH rises posteriorly, along the warmer iris and falls anteriorly, along the cooler inner surface of the cornea. During this descent, any particulates present in AH will often sediment out onto the corneal endothelium in the inferior half of the cornea (e.g. pigment in Krukenberg’s spindle or leukocytes forming keratic precipitates in uveitis). Ultimately, AH leaves the anterior chamber through two major outflow pathways, both of which originate in the irido-corneal angle of the eye. One of these pathways, termed the conventional or trabecular outflow pathway, is pressure-sensitive and handles the aqueous humor entering the angle anterior to the scleral spur. The remainder of the AH, entering the anterior chamber angle posterior to the scleral spur, enters the pressure-independent uveoscleral pathway.12
Anatomy of the ciliary body
The ciliary body, the middle portion of the uvea, plays roles in both aqueous production and outflow. The current discussion will be limited to its role in aqueous inflow. Situated between the choroid and the iris, the ciliary body is subdivided into two parts, the pars plicata anteriorly and the pars plana posteriorly.13 The pars plana appears to play no role in the production of aqueous humor. Consistent with its name, the inner surface of the pars plana is flat. The inner surface of the pars plicata, exhibits a ring of fin-like ridges surrounding the lens, each of which is termed a ciliary process (Figure 2). It is the ciliary processes that secrete AH. In meridional sections, the ciliary body appears as shown in Figure 3. Although rarely discussed as such, the pars plicata region of the ciliary body functions as an exocrine gland of the category known as a secretory sheet. Like other exocrine glands (e.g. lacrimal gland) it is covered by a secretory epithelium, beneath which is a fibrovascular connective tissue stroma and its secretion is regulated by autonomic innervation and hormonal factors. Unlike most other exocrine glands, the inner surface of the entire ciliary body is lined by two layers of epithelial cells, rather than just one. These layers are named for the presence or absence of melanin pigment. The layer closest to the underlying fibrovascular ciliary body stroma contains pigment (the pigmented ciliary epithelium-PCE) and the layer closest to the posterior chamber is devoid of pigment (the non-pigmented ciliary epithelium NPCE).13 Together, these two epithelial layers work in concert to secrete AH.
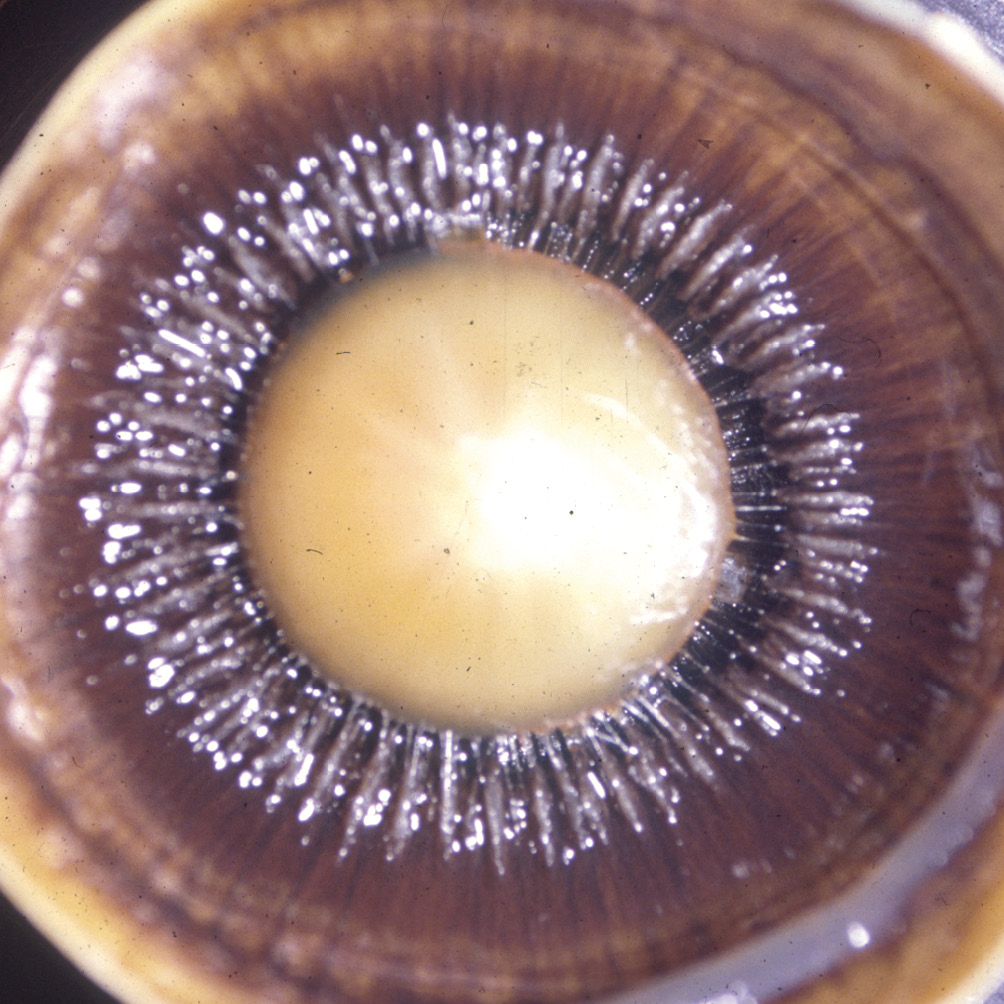
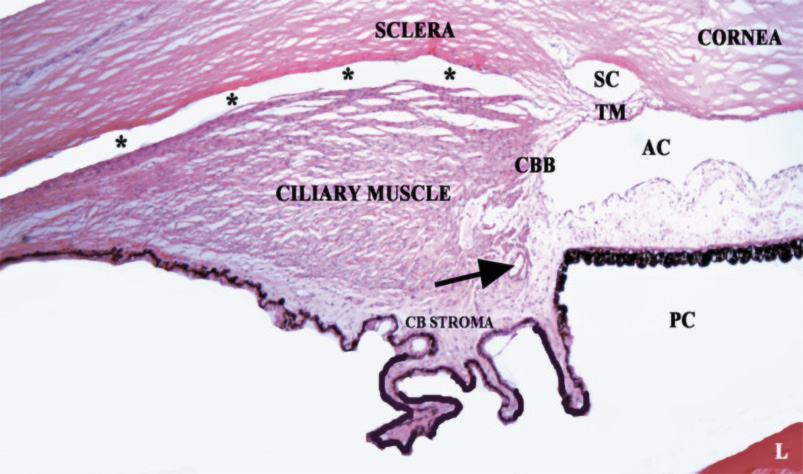
Production of Aqueous humor
The anatomical and physiological relationships involved in the production of AH, are most simply discussed as two steps, realizing that these two steps are only semi-independent. The first is the elaboration of a plasma ultrafiltrate from capillaries into the ciliary body stroma, and second is the formation and secretion of AH from this filtrate by the ciliary epithelial layers working in concert.14
Formation of the Ultrafiltrate by the Ciliary Body Microvasculature
The blood vessels within the fibrovascular core of each ciliary process arise from the major circle of the iris (Figure 2b). These vessels give rise to large capillaries that are of the fenestrated type. Fenestrated capillaries are highly permeable and will passively leak fluid, ions and even plasma proteins into the fibrovascular stroma of each ciliary process.15 These capillaries are sufficiently leaky that the concentration of plasma-derived proteins in the ciliary body stroma if nearly 75% of that found in plasma itself. The variables in controlling the amount of ultrafiltrate produced include the state of vasoconstriction, intravascular pressure vs intrastromal pressure (where intrastromal pressure is the same as IOP) and the relative concentrations of ions and proteins inside and outside of the vessels. Vasoconstriction reduces the amount of filtrate.16
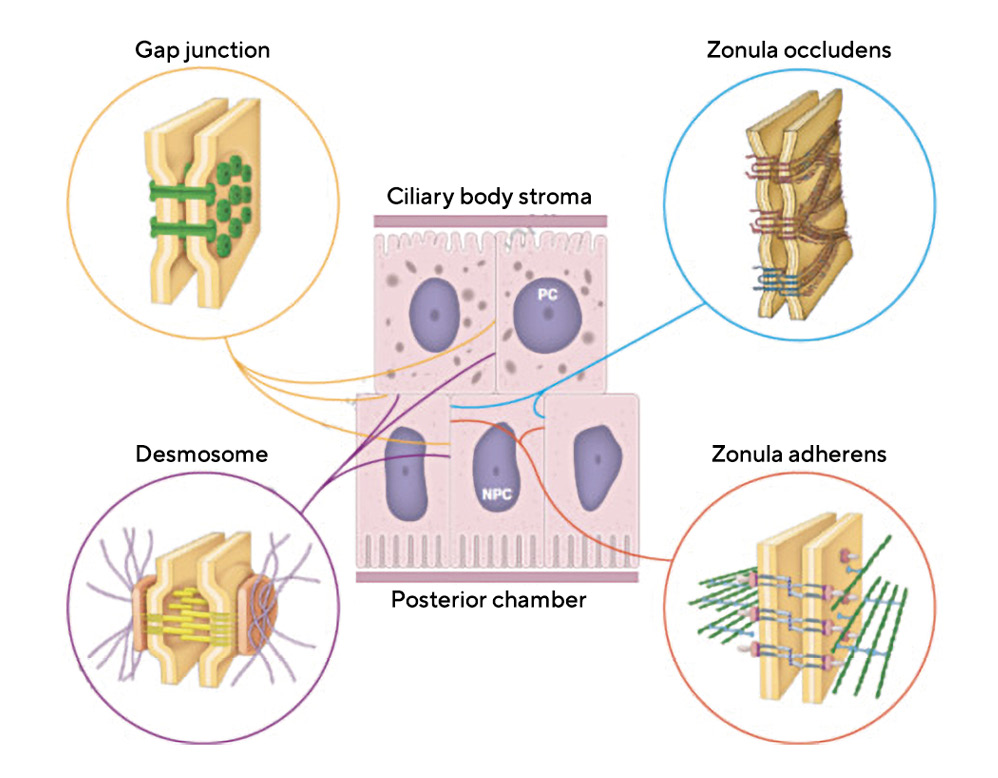
Secretion of Aqueous Humor by the Ciliary Epithelium
Prior to a discussion of the physiology of aqueous humor production, there are two membrane specializations within the layers of the ciliary epithelium that that are central to an understanding of AH – these are gap junctions and tight junctions.17 Additional intercellular junctions in the ciliary epithelium include desmosomes, which are multiple, focal junctions that tie the cellular cytoskeletons of adjacent cells together for stability. In addition, there is a zonula adherens junction that provides a single belt of cellular adhesion between only the non-pigmented ciliary epithelial cells (Figure 4).17
Gap Junctions
Gap junctions are focal membrane specializations, composed of proteins that are members of the connexin family. The junctions are formed by placing 6 connexins in a ring, within the membranes of adjacent cells. In each cell, these connexin rings, termed connexons, are placed at the same location so that they can be joined. Together, these joined connexons outline a hollow tube between the cytoplasm of the adjacent cells. This channel can be opened or closed based upon either membrane voltage levels or concentrations of certain ions such as calcium. When open, gap junctions allow for chemical messaging between cells in order for them to operate in a coordinated fashion. Gap junctions also provide low resistance passageways for electrical messaging. Numerous gap junctions exist between adjacent non-pigmented ciliary epithelial cells, between adjacent pigmented ciliary epithelial cells and between the pigmented and non-pigmented cell layers, where they are joined.17 These allow for ion transport between the two cell layers and provide a means of communication for coordination of the entire ciliary body to function like other secretory glands.18
Tight Junctions
Tight junctions (aka singular-zonula occludens, plural-zonulae occludentes) play little role in cell-to-cell adhesion. Their primary role is prevention of diffusion of macromolecules like proteins, between cells of an epithelial layer, whether that layer lines a blood vessel or is an epithelium at an internal or external body surface. This includes the epithelial surface of the ciliary body (Figure 4). As noted earlier, the protein content of AH in the anterior chamber is less than 1% of that found in plasma and yet the plasma protein concentration within the adjacent ciliary body stroma is almost 75% of that found in plasma.17 Proteins scatter light and create turbidity, degrading image quality in the eye. Additionally, the non-specific entry of plasma-derived proteins, such as albumin, can bring with it unwanted or harmful growth factors, and potential antigens deleterious to the special environment required within the eye. To prevent this, a selective barrier must be positioned between the ciliary body stroma and the posterior chamber. That barrier is formed by the series of tight junctions that seal the intercellular cleft between all of the non-pigmented ciliary epithelial cells. It is disruption of these tight junctions in anterior uveitis that results in the increased amounts of protein in the AH, referred to clinically as “flare”.17 Tight junctions also play a direct role in the secretion of AH as outlined below.
Overview of AH Formation from the Plasma Filtrate by the Ciliary Epithelium:
In it’s simplest form, it is the movement of sodium (Na+) and chloride (Cl-) ions (NaCl) from the ciliary body stroma into the narrow intercellular clefts between adjacent non-pigmented ciliary epithelial cells that drives aqueous secretion.18 Within these narrow clefts, sodium is concentrated to hypertonic levels, drawing water from cells through osmosis. This fluid in the intercellular cleft is prevented from flowing back toward the ciliary body stroma by the tight junctions and thus moves toward the posterior chamber in the form of aqueous humor. Additional water moves through water pores created by aquaporins.19 Energy for secretion is supplied by ciliary epithelial cell mitochondria as ATP, the product of aerobic metabolism of glucose. In its role facilitating secretion of AH, ATP is utilized by a Na+, K+ ATPase enzyme pump, predominantly localized in the basolateral plasma membranes of the NPE cells.18
The Na+, K+ ATPase Pump
The Na+, K+ ATPase protein within the cell membrane, has a pocket facing the cytoplasm of the cell. This pocket has an affinity for Na+ ions and can bind three of them. Once all three sites are bound, this prompts a molecule of ATP to bind the cytoplasmic side of the protein and attach one its three phosphate groups to the protein through a process called phosphorylation. Phosphorylation prompts a conformational change, flipping the protein such that the pocket becomes open to the outside of the cell. This also changes the affinity of the pocket from preferentially binding sodium to binding potassium. Thus, the three sodium ions diffuse out of the pocket and are replaced with two potassium ions from the extracellular environment. Once the two K+ ions are bound, this prompts dephosphorylation of the protein. Dephosphorylation prompts the protein configuration to revert to its original state, opening to the inside of the cell. In the process, two K+ ions are transported into the cell and the affinity of the pocket for Na+ ions is reestablished. With this pump, using the energy from one ATP molecule, three Na+ ions are moved out of the cell against their concentration gradient, in exchange for the movement of two K+ ions into the cell. This process does not create a build up of K+ ions in the cell because, as noted earlier, K+ ions, unlike Na+ ions, can diffuse back out through the cell membrane to a limited extent.
But, in the process of AH secretion, the Na+, K+ ATPase enzyme pump is not used solely to pump sodium ions into the intercellular clefts between NPCE cells. By pumping sodium ions into the intercellular cleft, the Na+, K+ ATPase pump also reduces the intracellular concentration of sodium ions in the cytoplasm of both the NPCE and PCE cells. This sustains a downhill concentration gradient in sodium and chloride concentration from the ciliary body stroma into the PCE and then the NPCE cells, ensuring that the concentration of these ions is always greater in the ciliary body stroma than in the cytoplasm of the ciliary epithelial cells. Doing so is critical to the mechanisms used by the PCE to import these ions into the cells from the stroma.14 These ion movements rely upon a complex array of co-transporters (symports), counter-transporters (antiports), facilitated-diffusion carriers (uniports), and ion channels that allow PCE cells to pick up ions from the ciliary body stroma and pass them from the pigmented to the non-pigmented ciliary epithelial cells through gap junctions. All of these ports are specific intramembranous proteins, floating in the lipid bilayer of the cell membranes. To function properly, these proteins must be restricted to only one surface of the epithelial cells or the other, ensuring that they move ions in the proper direction. Here again, tight junctions play a critical role in preventing these floating proteins from moving from the apical to the basal side of the these cells, where they could move ions in the wrong direction.
Uniports, Symports and Antiports in AH Secretion:
Some ions, like potassium (K+), can diffuse through cell membranes to a greater or lesser extent. In such cases, their concentrations inside and outside of cells will equilibrate, eliminating a concentration gradient from forming. Sodium ions, however, cannot diffuse through plasma membranes and hence, a large concentration gradient of sodium ions can be sustained across cell membranes. Importantly, this gradient can be put to work, moving not only additional sodium ions passively down this concentration gradient. Additionally, however, using special intramembranous proteins called ports, this sodium gradient can also be used to facilitate movement of other ions as well, sometimes even moving them AGAINST their concentration gradient, without expending cellular energy to do so. Ports are intramembranous proteins that fall into three main categories, uniports, symports and antiports. Opening and closing of ports is regulated either by voltage differences across cell membranes or by binding of specific molecules termed ligands.
- Uniports bind one molecule of a particular solute at a time and moves it across a membrane from a higher to lower concentration of that solute.
- Symports simultaneously move different molecules or ions across a cell membrane, both in the same direction. Typically, one ion will move passively down its electrochemical gradient, dragging the other molecule with it in a way that allows the second molecule to move AGAINST its concentration gradient toward an area of higher concentration, without expending energy to pump it against that gradient. In the case of aqueous production, it is usually the passive movement of Na+ ions from the higher concentration in the ciliary body stroma to the lower sodium concentration within PCE cells that allows another ion to enter the PCE cell against its concentration gradient.
- Antiports move two or more different molecules or ions across a cell membrane, but in opposite directions. Antiports are another passive way of moving solutes against gradients. As with symports, one ion moves down its electrochemical gradient. But unlike with symports, antiports move the second ion in the opposite direction AGAINST its own electrochemical gradient. Again, this happens without direct expenditure of cell energy in the form of ATP. These are not pumps.
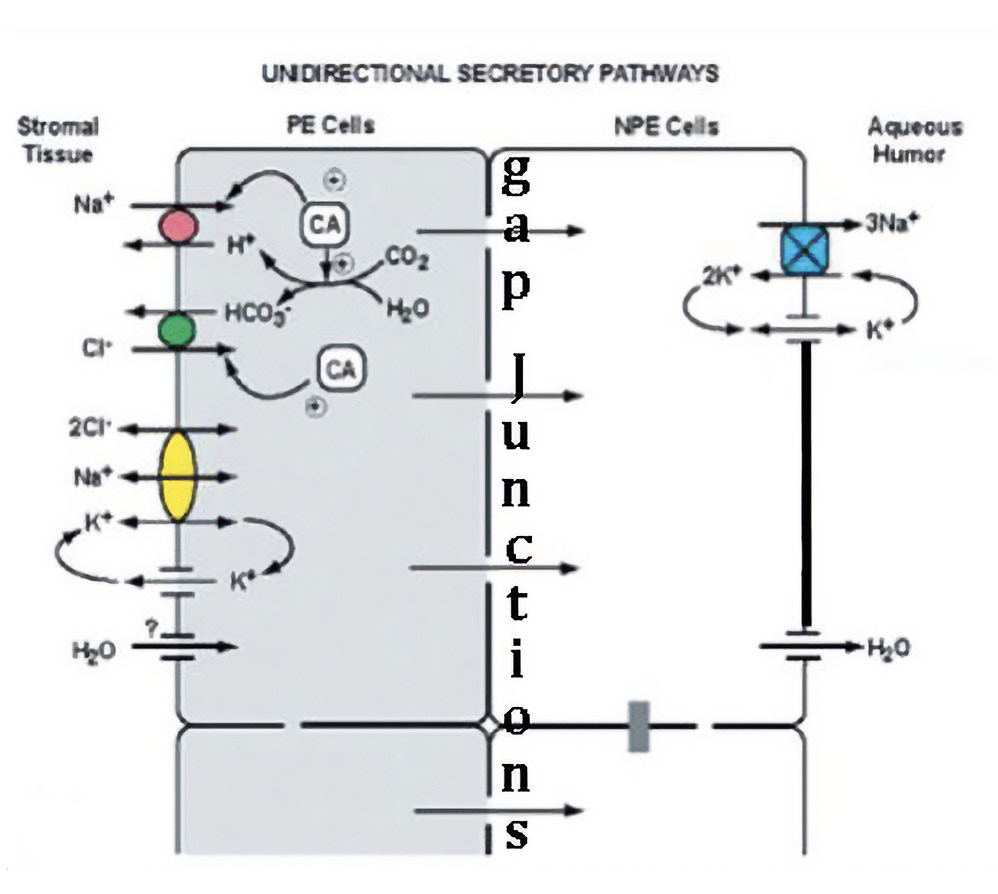
Sequential Steps in Ciliary Epithelial Secretion:
The source material from which AH is secreted is the reservoir of fluid, ions and macromolecules that have entered the ciliary body stroma via ultrafiltration through the fenestrated marginal capillaries within each ciliary process. AH secretion is initiated by the uptake of Na+ and Cl- ions through two sets of electrically neutral antiports within the membrane of the pigmented ciliary epithelial cells, facing the stroma.14, 18 Figure 5 shows a simplified version of this process, neglecting certain other port systems that supplement the movement of Na+ and Cl- into the PCE cells. Because the concentrations of Na+ and Cl- are lower in the PCE cell cytoplasm than in the stroma, these ions will move into the cell through ports along their concentrations gradients. But for each of these ions to come in, there must be a supply of another ion of the same valence that can move out. The cation exchanged for Na+ is hydrogen (H+) and the anion exchanged for Cl- is bicarbonate (HCO3-). Na+ enters the PCE cells through a port termed a Na+/H+ Exchanger (NHE1). Chloride enters the PCE through a port termed an Anion Exchanger (AE2). Using these ports to move ions of the same valence in the opposite direction, the charge differential on the inside and outside of the cell remains the same.14, 18 Clearly, for this system to work, there must be a continuous supply of hydrogen ions (H+) and bicarbonate (HCO3-) ions available in the cytoplasm, or these critical ion exchanges cannot occur. This continuous supply of H+ and HCO3- ions is accomplished using the enzyme carbonic anhydrase (CA) to convert cytoplasmic water and carbon dioxide into exactly the set of ions required for exchange, doing so through the intermediary carbonic acid (H2CO3) (Figure 4). The equations are as follows:
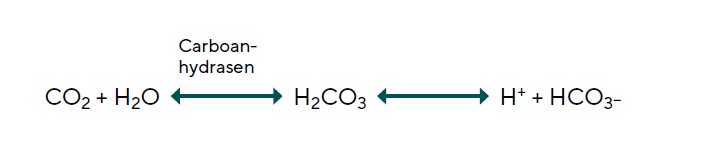
Given the critical role played by carbonic anhydrase in the uptake of the Na+ and Cl- needed to secrete aqueous, a decrease in the amount of enzyme available will reduce aqueous production.19 If aqueous outflow is not changed, this reduction in aqueous production will reduce IOP. Current evidence suggests that the carbonic anhydrase inhibitors used in the treatment of glaucoma likely reduce AH secretion and IOP by inhibiting the NHE-1 and AE2 antiports.18 The concentration of Na+ and Cl- are lower in the NPCE than in the PCE and so these ions continue moving down their gradient by flowing through the gap junctions that join the membranes of NPCE and PCE cell layers. The NPE cells transport Na+ to the narrow intercellular clefts of the NPCE, through their Na+ K+ ATPase pump and release Cl- through Cl- channels. The molecular identity of the Cl- channels remains unclear. The mechanisms underlying the movement of water are less well understood than those for transfer of ions. In addition to movement of water to dilute hypertonicity between NPCE cells, there are aquaporin water channels that facilitate release of water from the NPE cells into the AH.20
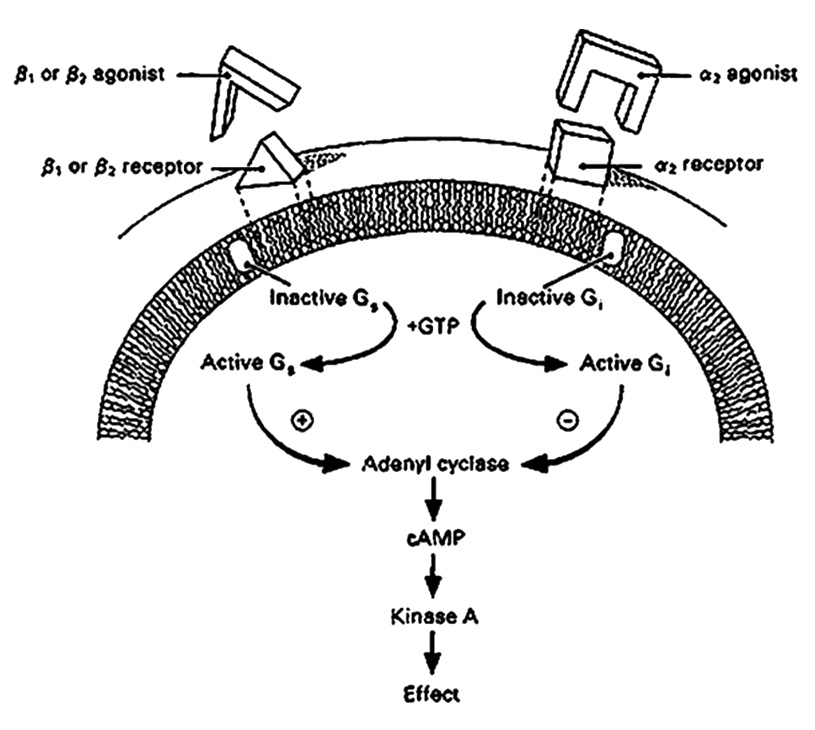
Receptor Systems Regulating Aqueous Production
Several receptors has been identified in the ciliary body of various species, including receptors for natriuretic peptides and others.21 In addition, a host of additional neuropeptides (e.g. vasoactive intestinal peptide, neuropeptide Y, opioids, and substance- P) have been identified within the tissues of the ocular anterior segment and these are under investigation as potential therapeutic targets to reduce aqueous production or outflow. Very heavily investigated are adenosine agonists and various nitric oxide donors.22 The latter of these can serve as nitrovasodilators in control of IOP, likely mediated via nitric oxide release.23-25
Beta Adrenergic Receptors The most thoroughly investigated receptor system known to affect aqueous production is the beta-adrenergic receptor. Beta-adrenergic receptors, both β1 and β2, have been localized to the ciliary epithelium.26-27 Binding and stimulation of these receptors initiates a signal transduction cascade of molecular events, beginning with activation of a regulatory intramembranous G-protein (Figure 6).28 In the case of β-adrenergic stimulation, the G-protein stimulates the cytoplasmic second messenger cyclic AMP, which has also been localized to the ciliary body.29 Cyclic AMP can pass through gap junctions, producing coordinated action among several cells. This, in turn, leads to phosphorylation of protein kinase-A and then an increase in AH secretion (Figure 6). What is currently unclear is the mechanism through which these events lead to the ultimate effect on AH inflow. It has at least been established that cyclic-AMP levels influence Na+, K+ ATPase activity.30 As cyclic-AMP levels rise, the Na+, K+ ATPase pump cycles more rapidly and more aqueous is produced. The converse is also true. Hence, β-antagonists (blockers) such as timolol maleate, lead to therapeutic reductions in both aqueous inflow and IOP.31 Most β-adrenergic antagonists (aka β-blockers) used in the treatment of glaucoma, are capable of binding both β1 and β2 receptors. β1-adrenergic receptors are located mainly in the heart and in the kidneys, while β2-adrenergic receptors are located mainly in the lungs, gastrointestinal tract, liver, uterus, vascular smooth muscle, and skeletal muscle. For patients with obstructive pulmonary diseases such as asthma, emphysema and COPD, β2-adrenergic blockers could worsen respiratory symptoms by limiting dilation of their airways. For such patients there are what are termed “cardioselective” β-blockers. These act primarily on β1 receptors, the type found in the heart. Their benefit in patients with pulmonary disease is that these drugs will not interfere with β2 receptors in the lungs. While cardioselective β blockers (β1) are safer to use in patients with pulmonary disease, they are less effective in reducing IOP per unit dose than the more commonly used mixed β1, β2 agents. Because beta blockers can also reduce the heart rate and force of cardiac contraction, beta blockers are also contraindicated in patients with sinus bradycardia (i.e. regular rhythm but a beat rate below 60 beats/minute) and those with heart block (i.e. delay in the timing of ventricular contraction relative to atrial contraction).
Alpha Adrenergic Receptors
Alpha2 adrenergic receptors are also present in the ciliary body.32 Like β receptors, they are also coupled through G-proteins to adenylyl cyclase and cyclic AMP, but in a negative fashion. This is because the G-protein to which they are coupled is inhibitory rather than stimulatory (Figure 6). So, in this case, it is the agonist that reduces AH secretion rather than the antagonist. (Figure 6).33 Alpha2 agonists are also available for use in glaucoma management. They appear to act through suppression of aqueous inflow without an apparent effect on outflow facility,33 although effects mediated via reductions in episcleral venous pressure and ciliary body vascular flow have been reported.34-36 Finally, as one might expect, β-blockers and a2 agonists can be used together to push IOP even lower, when the target pressure reduction is not achieved with monotherapy.
Conflict of interest
The author has no conflict of interest with regard to the methods and devices mentioned in the article.
COE Multiple Choice Questionnaire
The publication "The Role of the Ciliary Body in Aqueous Humor Formation" has been approved as a COE continuing education article by the German Quality Association for Optometric Services (GOL). The deadline to answer the questions is 01. November 2023. Only one answer per question is correct. Successful completion requires answering four of the six questions.
You can take the continuing education exam while logged in.
Users who are not yet logged in can register for ocl-online free of charge here.